Einstein's Spooky Action At A Distance Is All Around Us
Classical physics are based on Newtonian laws. According to popular legend, Isaac Newton was inspired to develop his theory of gravity when he observed an apple falling from a tree and while this story is a simplified version of the event, the 16th-century tale symbolizes Newton’s insights into gravity and motion and how physical objects are deterministic. In addition to his work on gravity, Newton made significant contributions to mathematics, including the development of calculus, which he formulated to solve problems in physics and astronomy.
Now, the world we live in and play with is full of Newtonian mechanics. Large objects, galaxies, planets, meteors, people, animals, rocks and the interactions of all objects in any space are governed by the laws of Newtonian physics, widespread intuition and common sense, but this sense doesn't exist on certain scales.
At atomic and subatomic scales, a different set of laws come into play called quantum mechanics. These principles are counterintuitive and still not fully understood. They command the behavior of particles at these minuscule levels. Despite their mysterious nature, these fundamental laws are what make the existence and behavior of the large objects around us possible.
They're called atoms and each type of atom contains a unique number of subatomic particles: positively charged protons, neutral neutrons, and negatively charged electrons. The electrons move around the nucleus at astonishing speeds. These particles unite in precise configurations to form the diverse elements that make up the universe. Yet, despite their incredible speed, electrons don’t physically travel around the nucleus in a traditional sense—they mysteriously just show up within specific energy levels, again directed by an misunderstood set of rules.
Rules come from complex experiments and there are many that oversee how we perceive the universe. One key experiment that challenged classical ideas is the double-slit experiment, where light passes through two closely spaced slits and creates an interference pattern of alternating bright and dark areas, known as maxima and minima. This pattern is a hallmark of wave behavior, showing that light can spread out and interfere with itself. However, when the experiment is conducted with individual photons—particles of light—these photons still create an interference pattern over time, indicating that each photon behaves as both a wave and a particle. Yet, if we try to observe which slit the photon passes through, the interference pattern disappears, and the photons behave purely as particles. This experiment reveals that light behaves as both a wave and a particle, challenging the notion of symmetry in ways that become apparent at much smaller scales, as we'll explore.
Understanding Symmetry
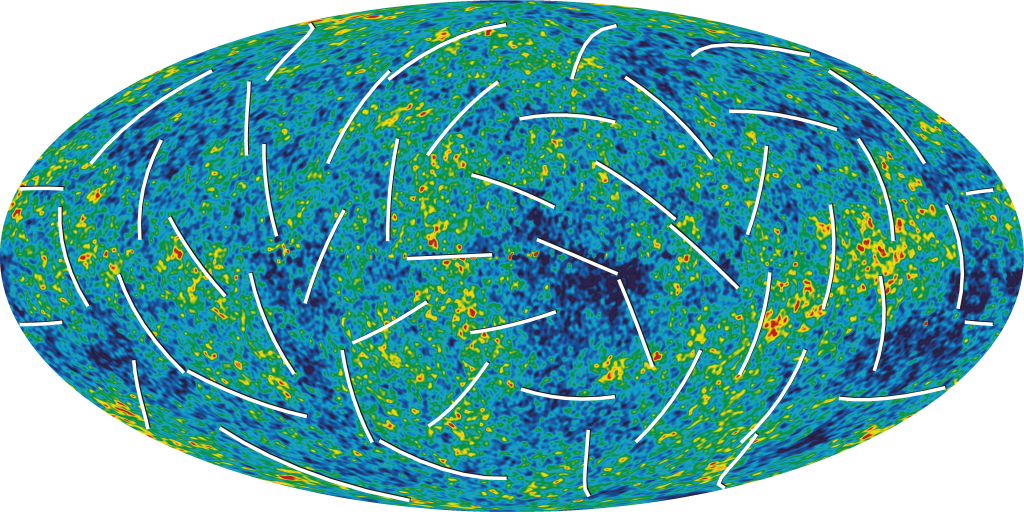
In mathematics, symmetry refers to a transformation that leaves an object unchanged. Think of it as a law that applies both in classical and quantum physics. For example, consider a spherical ball, which looks the same from any direction due to its symmetry, or an infinite rope, which remains unchanged no matter how far you go along it.
The universe itself exhibits symmetries because it follows these kinds of transformations. To visualize this, let’s imagine hopping to another universe. In this empty universe, if I throw a ball in any direction, it will never change its speed, direction, or rotation—conserving its momentum, angular momentum, and its energy.
Now, let’s return to our own universe, where perfect symmetries are disrupted by the presence of celestial and terrestrial bodies. These massive objects, whether drifting alone or clumped together, curve space and time, creating asymmetries that affect the speed, direction, and rotation of any object passing near them. This curvature of spacetime is why objects fall to Earth.
While the curvature of spacetime affects the motion of physical objects, light behaves differently—except in the extreme case of a singularity. One striking example of this is redshift, which can be likened to the stretching of a rubber band—the farther light travels through expanding space, the more its wavelength elongates, shifting towards the red end of the spectrum. This redshift occurs as the universe expands, stretching the wavelengths of light from distant objects over time. As this expansion continues, light from these objects shifts to longer wavelengths, making them appear redder and less bright.
As the universe continues to expand, the mathematical rules that govern symmetry begin to change subtly. Global symmetry, which once held everything in balance, gradually weakens over time. In the distant future, this weakening could mean that the light from far-off objects will stretch so much that it becomes invisible, even to our most powerful telescopes. As the universe keeps expanding, our view of the cosmos will slowly dim, leaving us with only the closest stars and galaxies to observe.
That’s a bit depressing, so let’s shift to a different but closely related topic that might cheer you up. This one is another type of symmetry—a universal invariance. If global symmetry is the skyscraper, then gauge symmetry is the sky, stretching endlessly and touching everything, everywhere.
One of the fundamental forces of the universe is electromagnetism, alongside the Fine Structure Constant. Like the Four Heavenly Kings, it’s part of an important quartet that physicists consider among the most mysterious attributes of the cosmos. Light comes and goes—it brightens and dims. But electromagnetism is all around us, the most influential of the four forces, making the physical world possible. It governs how water behaves, shapes the atmosphere, and ensures your phone doesn’t slip through your hand. Everything we see and interact with owes its existence to the specific characteristics of electromagnetism.
Electromagnetism is one of the most studied topics in physics, yet it still isn’t fully understood. What we do understand, though, are the interactions of the subatomic particles that generate electromagnetism. These interactions have been active since the Big Bang, never deteriorate, and remain constant throughout all that was and all that will be. I’m talking about electrons, and I’m here to tell you all about them. By the end, you might have an entirely new perspective on traveling through space and time.
Atomic Behavior
Atomic behavior is unlike any process we can observe in the universe. These processes are so peculiar and strange that even experts find it hard to understand them without injecting imagination into their theories and experiments. Human intuition, after all, is based on the large, small, telescopic, or microscopic objects we see around us—objects whose behavior we can often predict with some accuracy. That same intuition falls short in the quantum realm—it’s like looking at abstract art. Processes on that scale are just different but, let’s try to build an understanding this way: “Big fleas have little fleas upon their backs to bite them, and little fleas have lesser fleas, and so ad infinitum.” - Jonathan Swift, author of Gulliver’s Travels.
Jonathan Swift’s observation about fleas biting fleas is a fitting metaphor for the quantum world, where particles within particles interact in ways that challenge our understanding. Just as big fleas have little fleas upon their backs, atoms have smaller components, and those components have even smaller parts, each having by its own set of rules.
We see this pattern everywhere in the universe. From the elegant arms of spiral galaxies to our orbit around the Sun, down to adenine, thymine, and the rest of the molecules that make up DNA, nature forms large structures from collections of smaller components. The smaller we scale, the smaller the components become. Scale enough, and we’ll reach the atom—the most fundamental unit of matter. Understanding their structure reveals the intricate building blocks of everything we know.
Atoms were once thought to be indivisible and overall neutral—a concept first proposed by John Dalton in 1803. However, this misconception was corrected by the cathode ray experiment, which revealed that atoms are composed of subatomic particles. Positive protons and neutral neutrons clump together to form a nucleus, while negatively charged electrons 'orbit' around it.
Like a Fruit Pudding?
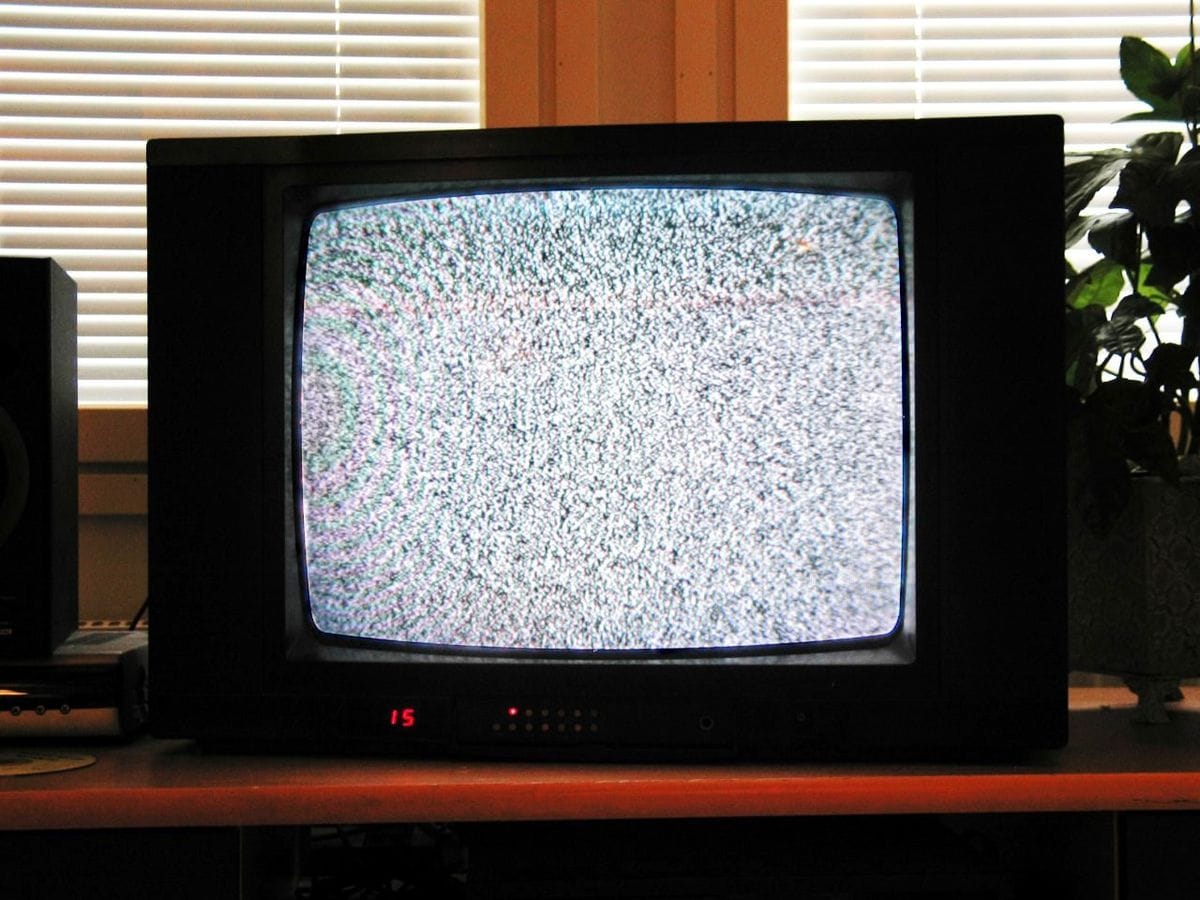
Electrons are to chemists what dogs are to humans—the most interesting part of any element because they’re free to move around the nucleus of an atom. Erwin Schrödinger’s model, published in 1926, predicts where these particles are most likely to be found around the nucleus. They appear—an important word in this context—with ferocious unpredictability, moving like rodents scurrying in the dark. Like rats below deck on a dark night, you never know where they are until you turn on the lamp, or in this case, measure the atom. Broader, less detailed measurements had been made since 1847.
In the 1870s, William Crookes made remarkable strides in understanding cathode rays through groundbreaking experiments. He showed that these rays traveled in straight lines, carried momentum, and could be deflected by magnetic fields. Crookes observed fascinating phenomena, such as the greenish glow on glass and the turning of a paddle wheel, suggesting that cathode rays were composed of particles. However, despite these significant findings, Crookes remained puzzled by the nature of these rays, unsure of what they were made of. This uncertainty paved the way for J.J. Thomson’s identification of the electron in 1897.
Thomson’s experiment involved a simple glass tube with two electrodes: the cathode, which is negatively charged, and the anode, which is positively charged. These electrodes were connected to a high-voltage power source. When activated, the cathode emitted a stream of particles known as the cathode ray, which traveled from the cathode to the anode. A small hole in the anode allowed the beam to continue in a straight line along the tube.
Thomson then placed two oppositely charged plates at the top and bottom of the tube to see if they would affect the beam’s direction. He noticed that the ray bent toward the positively charged plate, showing that the particles in the beam were negatively charged. This observation led Thomson to identify the particles as electrons. By analyzing the curvature, he estimated the charge-to-mass ratio of these electrons, revealing their surprisingly small mass.
Thomson’s experiments with cathode rays allowed him to measure the mass, speed, and charge of electrons. These findings led to a new model of atomic structure: the plum pudding model. In this model, the atom is likened to a plum pudding, where the 'pudding' is a positively charged substance, and the 'plums' are the negatively charged electrons scattered throughout. This model suggested that atoms consist of electrons embedded in a positively charged 'soup.'
Thomson’s work also laid the foundation for Cathode Ray Tube (CRT) technology, which led to the development of early television. You might remember the bulky component cables colored red, green, and blue that carried video signals for older TVs and monitors. Inside these CRTs, electron guns corresponding to each of these colors shot beams of electrons toward the screen. Magnetic fields guided these beams across the screen, combining them to create the full-color images we used to see.
Another thing we noticed with older TVs was the static or ‘snow’ that appeared when there wasn’t a signal. This static came from random electronic noise and interference, including contributions from the cosmic microwave background. While this wasn’t a direct view of electrons, it showed the chaotic, uncontrolled activity within the TV’s circuitry. Even though we can’t see electrons with the naked eye, the randomness of these beams created a visual effect that hinted at the underlying quantum behavior of particles.
The static was mostly due to the breakdown of the system that directed electron beams across the screen. But the complexity of the static also came from the inherent unpredictability of subatomic particles, particularly electrons, which behave in probabilistic ways. This randomness contributed to the chaotic appearance of static on the screen.
Without realizing it, this gave everyday consumers a glimpse into the unpredictability of the quantum world. While the electron itself was discovered in the late 19th century, it wasn’t until the early 20th century that scientists began uncovering even more mysterious properties, like quantum spin. One of the most important experiments that led to this discovery was carried out by Otto Stern and Walther Gerlach.
'Spin' by Otto Stern and Walther Gerlach
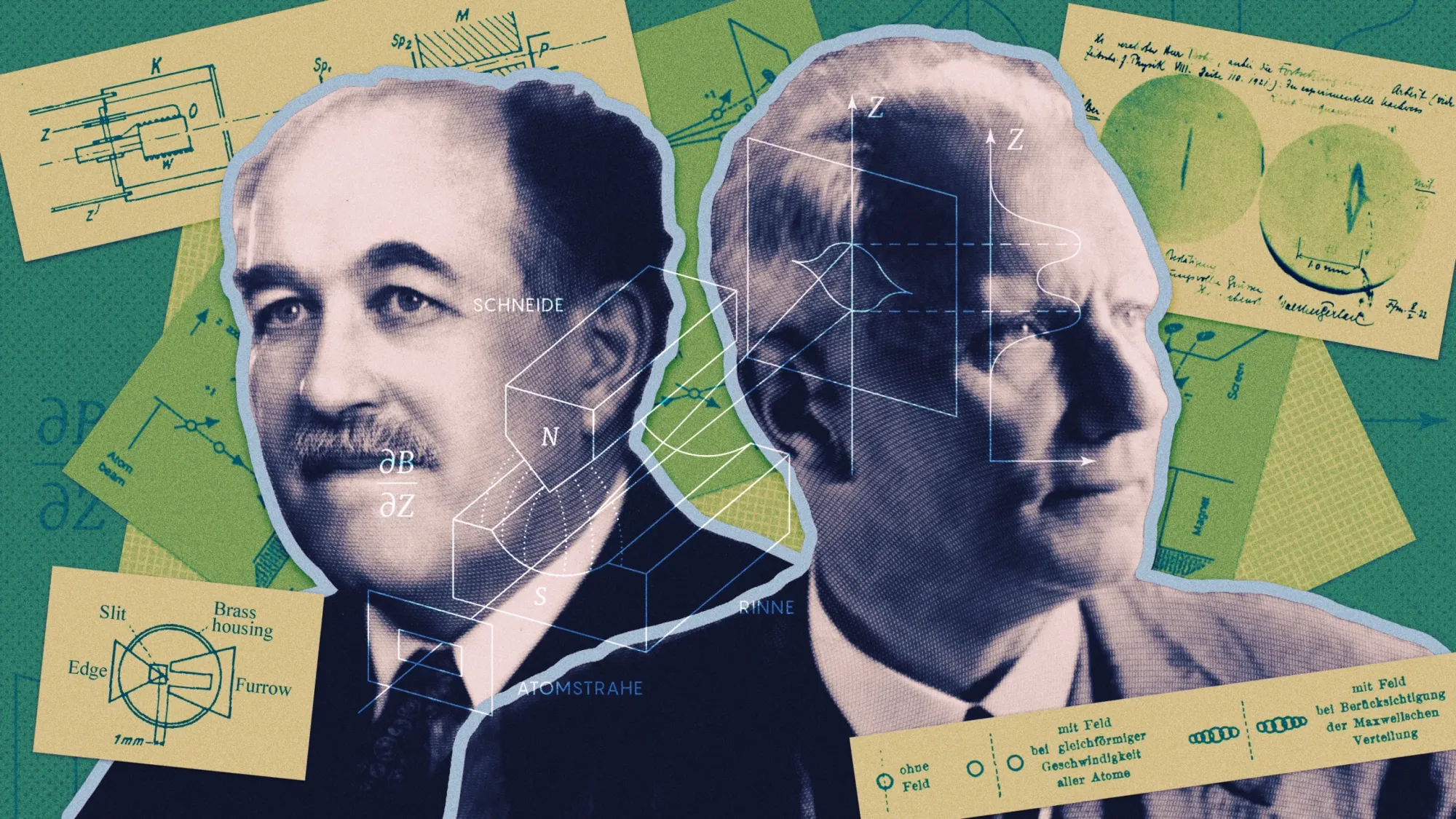
In the winter of 1922, German physicists Otto Stern and Walther Gerlach made a groundbreaking discovery with their famous Stern-Gerlach experiment. This experiment provided the first direct evidence of quantum spin, a fundamental property of subatomic particles. Designed to test the hypothesis of quantized angular momentum, the Stern-Gerlach experiment was a pivotal moment in the early 20th century, a period when theories about quantization were rapidly gaining traction. This was particularly significant following Albert Einstein’s theory of general relativity and Niels Bohr’s 1913 model of the hydrogen atom, both of which suggested that electron energy levels are quantized.
To grasp the concept of quantization, imagine water flowing from a faucet. While it appears continuous, it’s actually composed of discrete molecules of hydrogen and oxygen when closely observed. Similarly, quantum mechanics reveals that atomic systems, once thought to operate like miniature solar systems with planets orbiting continuously, actually work in quantized steps. Picture if, in our solar system, giving Mercury just the right amount of energy instantly moved it to Venus’s orbit, or boosting Jupiter’s energy caused it to leap to Saturn’s orbit. In quantum mechanics, electrons don’t move gradually between orbits; they jump between fixed energy levels when they absorb or emit specific amounts of energy. If given too much energy, they can be ejected entirely from the atom.
This analogy ties into the broader concept of quantization, where energy behaves like discrete packets. Picture a staircase: with just enough energy, you can jump up one stair. With more energy, you can jump two stairs, and with even more, you might leap several at once. The energy used in each jump is quantized—it comes in specific, discrete amounts. In quantum mechanics, particles like electrons absorb or emit energy in these fixed quantities, known as quanta. On CRT TVs, these electrons transfer their energy to excite phosphors on the screen, which emit light in specific colors, creating the images we were able to see.
So, just as stairs can be thought of as the 'quantum' units of a staircase, raindrops are the quantized units of rain, grains of sand are quanta of a beach, and each person is a quantum of the human race. These are all smaller components that collectively make up a whole.
When J.J. Thomson measured the electron, he discovered it had mass and, therefore, momentum. But was this momentum angular—were these particles spinning like tiny Earths on their own axes? This was the question that German physicists Otto Stern and Walther Gerlach set out to investigate. They designed an experiment to determine whether atoms, and by extension electrons, exhibited this kind of angular momentum. Using a radiation furnace, known as an Atomstrahlofen, they generated a beam of silver atoms and passed it through an inhomogeneous magnetic field. Their goal was to observe whether the atoms’ paths would split in a way that indicated quantized angular momentum—a groundbreaking step in understanding the fundamental properties of matter.
Given the experiment’s design, it was initially reasonable to assume that the magnetic moments—akin to tiny bar magnets—of the silver atoms would be randomly oriented, leading to a random distribution of deflections based on their orientation. However, the experiment produced a surprising result: instead of a continuous spread, the beam split into two distinct parts, corresponding to two possible states—'spin up' and 'spin down.' This outcome provided concrete evidence of the quantized nature of particles, showing that each atom’s spin was confined to one of these two discrete states, with no in-between—at least not while you’re looking.
Not only did the experiment demonstrate the quantization of spin, but it also represented a crucial advancement in our understanding of quantum mechanics. It revealed that even seemingly random aspects, such as the orientation of atomic spins, are dictated by the fundamental principles of quantization.
Building on the understanding of quantized spin from the Stern-Gerlach experiment, it’s important to recognize that spin is an intrinsic characteristic of all particles. This means it is an inherent property that defines the particle’s fundamental nature.
To fully grasp what it means for spin to be an intrinsic characteristic, we need to delve into quantum field theory—specifically, group theory, and even more specifically, back to symmetry. This is where things start to get a bit weird, but don’t worry, we’ll navigate through it together. Let’s stay focused.
Now, let’s address a common misconception: electrons don’t really have mass—or at least, they barely do. Yes, Thomson measured their charge-to-mass ratio, but their physical mass is almost negligible. While protons and neutrons, which make up the nucleus, are composed of even smaller particles called quarks, electrons belong to a family of fundamental particles known as leptons. As elementary particles, leptons like electrons aren’t made up of smaller components—at least, nothing that scientists can currently see or understand.
In quantum theory, electrons are treated as 'point-like particles,' meaning they have no size in classical terms. Yet, they do have mass, and their effects are real, much like how gravity pulls you toward the Earth without occupying space.
Electrons exhibit intriguing properties, like being in superposition—where they can exist in multiple states at once—and showing both wave and particle characteristics. Despite not spinning in the traditional sense, they still have angular and linear momentum.
Another strange phenomenon is quantum entanglement, where particles seem to communicate instantly, defying the limits of light-speed. But before we explore that, let’s first understand where electrons are most likely to be found around a nucleus.
What Are Orbitals?
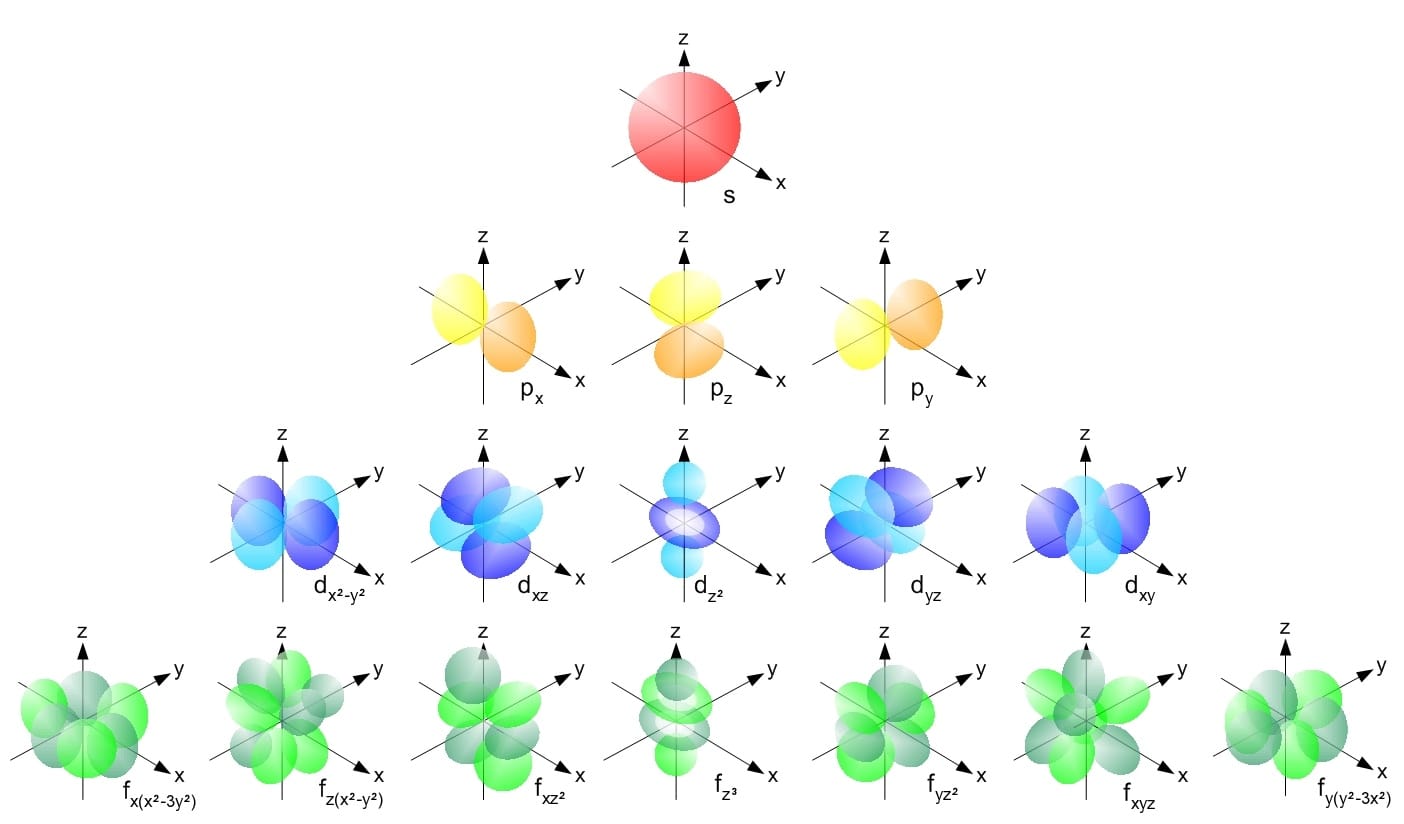
Now that we’ve explored the fundamental nature of electrons, let’s shift our focus to how they behave within an atom. Think of orbitals as dense clouds surrounding the nucleus, showing us where electrons are most likely to be found. These clouds offer insight into how active or energized the electrons are. In our model, electrons tend to stay close to their parent nucleus, but they can occasionally venture farther out. The further away from the nucleus you go, the less likely you are to find an electron, though the possibility never completely disappears. This understanding helps us predict how electrons behave and interact within an atom. So, now that we have a sense of what orbitals look like, how do they work?
Orbitals are regions around an atom’s nucleus where we’re most likely to find its electrons. Unlike physical paths, such as planets orbiting the sun, orbitals are more like zones or clouds where electrons tend to reside. These zones are described by Schrödinger's equation, which uses numbers to define the electron’s energy level, the shape of the orbital (like a sphere or a dumbbell), and its orientation in space. For example, some orbitals are spherical like a ball, while others have shapes resembling a dumbbell or more complex forms. The way electrons fill these orbitals—starting from the ones closest to the nucleus and moving outward—determines an element’s chemical behavior, how it bonds with other elements, and even its placement on the periodic table.
Unlike the particles that make up nuclei, which have some degree of uncertainty, the locations of electrons in an atom are governed by a working equation that shows us where the electron is likely to be found. Electrons can exist in multiple locations within their orbital at once, a concept known as superposition. This state only 'collapses' to a specific location when the electron is observed or measured.
The Superposition
To understand superposition, let’s combine the previous ideas of CRT static with the up or down spin of electrons. We know that when uncontrolled, electrons display erratic behavior and can exist in two possible states: up or down.
Now, here’s a thought experiment. Picture an electron with two characteristics: spin (which can be either ‘up’ or ‘down’) and ‘color’ (standing in for another quantum property, like energy state, which can be ‘black’ or ‘white’). We have an ‘up-down’ box that sorts electrons by their spin. When we send 1,000 electrons into this box, 500 come out with ‘up’ spin, and 500 with ‘down’ spin. But here’s where it gets strange. If you take those 500 ‘up’ electrons and pass them through a ‘color’ box, instead of all being the same color, half come out ‘black’ and the other half ‘white.’
Now, send those 250 ‘black’ electrons through the ‘up-down’ box again. Surprisingly, you get 125 ‘up’ and 125 ‘down.’ Didn’t we just sort these? Welcome to the bizarre world of quantum mechanics, where particles can exist in multiple states at once—a phenomenon known as superposition. Their properties only become definite when measured.
To further illustrate this unpredictability, imagine opening the box of a new phone, marketed as the ultimate gadget, only to find a slab of dirty clay inside. Disappointed, confused, appalled? Yes, but in the quantum world, this kind of unpredictability is the norm. Superposition challenges our everyday logic, making it seem as though electrons are constantly switching states when we’re not looking.
Now, let’s revisit quantum entanglement. For this to make any sense, we'll have to leave contemporary logic behind for a moment.
Imagine two people start at opposite ends of a magical oasis pool. One person has a bottle filled with divine water, and the other’s bottle is empty. They then run in opposite directions across the desert, without knowing if their bottle contains water. As soon as one of them stops and drinks from their bottle, the state of the other person’s bottle is instantly known—if the first person drinks, the other’s bottle instantly becomes full, no matter how far apart they are. This reflects how measuring one entangled particle instantly determines the state of the other. This phenomenon is known as quantum entanglement, and it makes light-speed look like child’s play.
Albert Einstein famously rejected the idea of quantum entanglement, referring to it as 'spooky action at a distance.' He believed that before two particles separated, they shared information that predetermined their states, making any further communication between them unnecessary.
However, Einstein's skepticism was ultimately proven wrong. Experiments conducted decades later, most notably by physicist John Bell in the 1960s, showed that entangled particles do not simply share pre-existing information. Instead, the act of measuring one particle instantaneously affects the state of the other, regardless of the distance between them. This confirmed that quantum entanglement is real and defies classical explanations.
Does Teleportation Come Next?
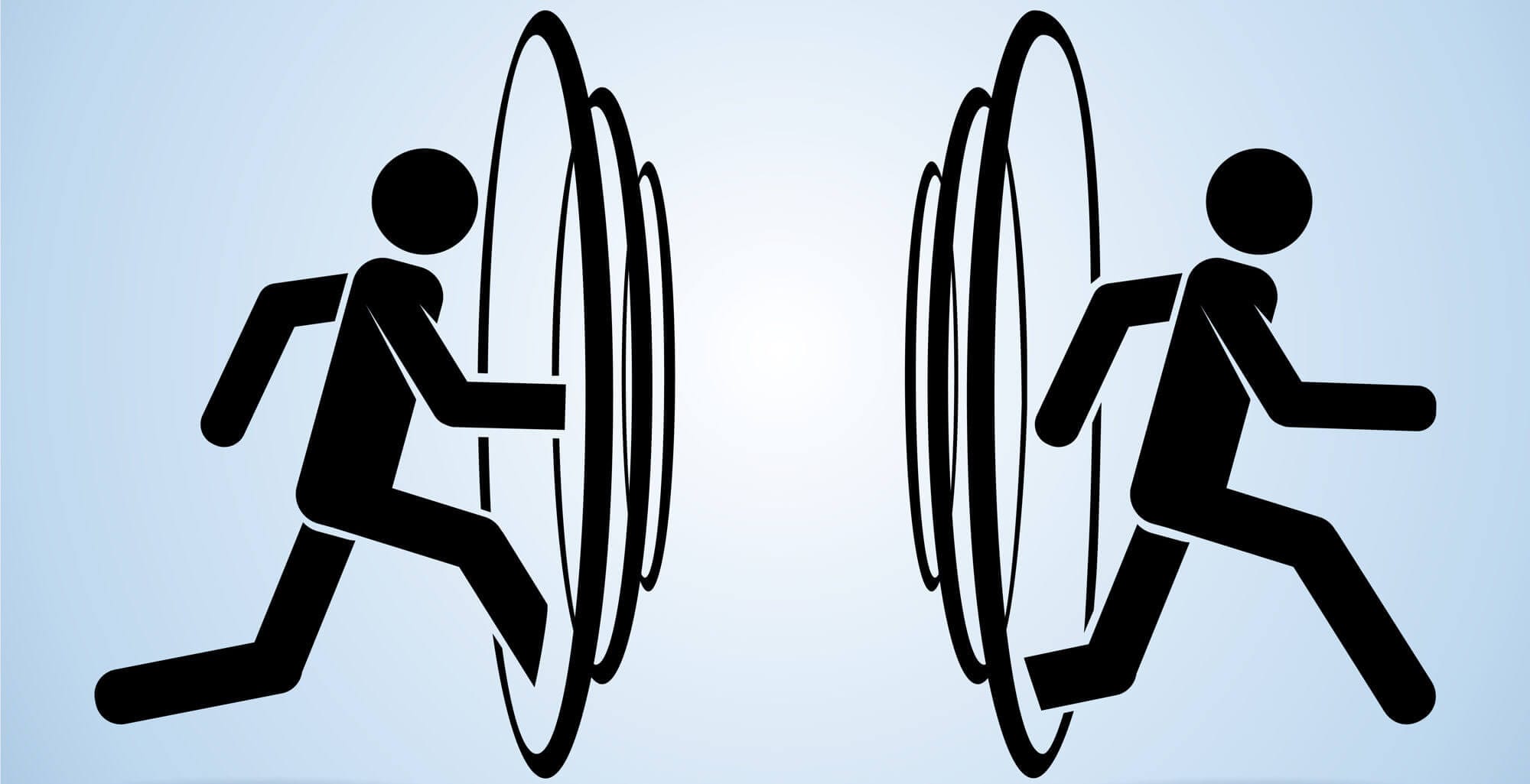
We’re already using the quantum world in different ways to advance our classical technology. Understanding that the language of computers consists of 1s and 0s, utilizing superposition allows for extremely fast computational speeds, which I’ll explore further in a dedicated section on quantum computing.
A physicist named Ian Walmsley at the University of Oxford led a team that successfully demonstrated how the strange behavior of quantum theory can also apply to larger objects.
Exploiting both superposition and entanglement, we arrive at quantum cryptography (Quantum Key Distribution). Quantum cryptography uses these unique quantum properties to create secret keys that only the intended recipients can know. If anyone tries to intercept the communication, the connection between the particles is disturbed, immediately alerting the parties involved. This makes quantum cryptography one of the most secure methods available today. Some banks, government agencies, and large corporations have already begun using QKD systems to protect highly sensitive data, with this encryption method expected to become more widely available in the near future.
But what about larger, non-computational objects? In a fascinating study published in Science in 2012, researchers explored the possibility of creating and observing quantum entanglement in large objects, specifically using diamonds at room temperature.
The experiment involved creating vibrations within the diamonds using laser pulses and then detecting specific photons emitted as a result. By analyzing these photons, the researchers found strong evidence that the vibrations in the two diamonds were indeed entangled. This discovery suggests that quantum effects, traditionally observed only in tiny particles like electrons or photons, can also occur in larger, more ordinary objects under the right conditions.
This finding challenges our traditional understanding of the boundary between the quantum world and the everyday world. It opens up new possibilities for technology, such as quantum computers or advanced communication systems, by demonstrating that quantum effects can be harnessed in larger, more practical systems.
Now, what about teleportation? Quantum teleportation, once a science fiction fantasy, is now a reality—though currently limited to small particles like photons. Unlike the fictional teleportation of objects or people, real quantum teleportation transfers the quantum state of a particle from one location to another using quantum entanglement. Here, the state of one particle instantly affects the other, regardless of distance.
The current success rate of quantum teleportation is around 25%, limited by the difficulty of performing a complete Bell-state measurement—a process that reveals how two entangled particles are connected. Researchers are working on ways to improve this efficiency, such as having the quantum state "piggyback" on an entangled photon, and have also demonstrated teleportation of continuous quantum features like squeezed states of light. These advancements expand the potential applications of quantum teleportation.
Scaling quantum teleportation to larger objects remains a significant challenge due to the need for entangled pairs and the prevention of decoherence, which disrupts quantum states. While teleporting large objects is currently unfeasible, the technology for teleporting individual atoms and possibly molecules is within reach, with significant implications for quantum computing, where teleportation could transfer quantum information between processors.
Quantum teleportation challenges our classical understanding of reality, echoing the philosophical debates between Einstein and Bohr. While Einstein was skeptical of "spooky action at a distance," Bohr emphasized the need to consider the entire quantum system. These experiments show that quantum mechanics is not just about describing nature but understanding what we can observe and measure in the quantum world, offering new insights into the nature of reality.